Oct. 26, 2006 Research Highlight Chemistry
Use salt for rich electronic states
Anion radical salts turn out to be a tasty treat for scientists
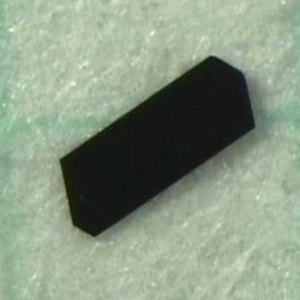
The world of condensed matter physics is full of intriguing effects that occur as a result of electrons interacting with each other. Well-known examples are magnetism and superconductivity. These effects were typically first observed in inorganic crystals and only later discovered in organic compounds. For example, superconductivity, the lack of any resistance in the electronic current flowing through a material, was first discovered in 1911, whereas it was only in 1980 that superconductivity has been shown for synthetic molecules.
The observation of such fundamental physics effects in organic molecules is not only exciting for scientists, but presents an intriguing possibility for future applications. Conventional electronic devices such as silicon transistors are fabricated on a nanoscale in a ‘top-down’ approach that relies on external technology. However organic molecules form naturally in a ‘bottom-up’ approach when presented with the right external conditions. Scientists are hoping that it will eventually be possible to use self-assembled organic molecules in electronic devices, or even as entire integrated circuits capable of performing complex mathematical operations.
Designer molecules
In recent years, the field has seen significant advances in the understanding of the physics of artificial designer molecules. This had led to an increasing complexity and enhanced functionality of newly designed salts. One such family of molecular compounds are the anion radical salts based on the Pd(dmit)2 molecule (Fig. 1), studied by a team of materials scientists from the RIKEN Discovery Research Institute in Wako.
The salts exhibit a large variety of fundamentally different electronic states that is astounding. “The Pd(dmit)2 system is a unique playground that contains rich physics,” says Reizo Kato, whose team has been intensively investigating these salts. “It is an exciting challenge to understand the subtle behaviors of this molecular system.”
Most of these effects are rooted in the crystal structure of the salts. The structure of crystals can be determined using X-ray diffraction imaging, where the intensity distribution of X-rays diffracted from a crystal is directly related to their structure. In previous experiments, Kato’s team has shown that in Pd(dmit)2 salts the anions arrange themselves into strongly coupled pairs, known as dimers, which in turn form a layered structure with a triangular lattice (Fig. 2). The combination of the Pd(dmit)2 anion and various cations is crucial and determines the conducting properties of the salts in addition to external parameters such as temperature and pressure.
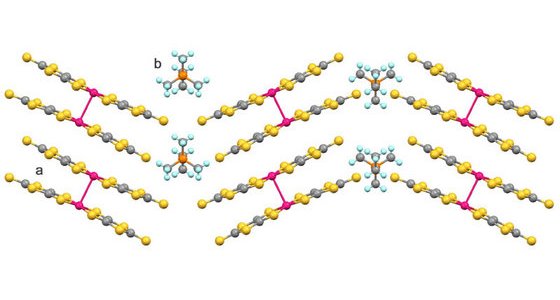
Small changes, big consequences
In their latest study, published in the Journal of the American Chemical Society1, the team investigates the new system that contains EtMe3Z+ (Z=P, As) cations. Compared with previously studied cations, the EtMe3Z+ cations have a more drastic influence on the electronic properties of the system. “Surprisingly, in the molecule-based conductor, the insulating cation is not a spectator and its geometrical and physical properties strongly affect the conducting properties of the whole system. In that sense, all components are important and the molecular system is a stage where everyone must play a part,” explains Kato.
As the RIKEN team discovered, the difference in the crystal structures of the salts and their electronic states is solely determined by a single atom Z in the cation, being either Phosphorus or Arsenic. “We did not expect that such a small change would lead to extraordinary differences in magnetic properties,” confesses Kato. At low temperatures, the salt with EtMe3As+ cations is antiferromagnetic, while that with EtMe3P+ is non-magnetic. However, the two salts also share similarities. At room temperature, both salts conduct poorly and when hydrostatic pressure is applied they become metallic. Upon cooling under pressure, both turn superconductive.
The structural relationship between these salts and the similarities in some of their electronic properties suggest a unified framework. The RIKEN team shows that this is a direct consequence of the layered crystal structure and down to variations in the interaction between the dimers on the triangular lattice. For the two salts, the arrangement of how the dimers are packed into the layers (Fig. 2) varies slightly. This leads to changes in the electronic interactions between the dimers and as a consequence to the suppressed antiferromagnetism in the salt with the EtMe3P+ cation.
The dawn of a new molecular age?
The large variety in the electronic properties of these salts from introducing only subtle changes to their composition has convinced Kato that “these findings will open the way to a new solid-state molecular science”. The flexibility with which the electronic states can be predetermined via the design of the molecules suggests new pathways to prepare well-defined electronic states. This could enhance our understanding of the fundamental physics governing these molecular systems.
In addition, it is possible to envisage the use of such molecules for novel applications and silicon technology provides yet another analogy to compare those two technologies. Replacing atoms in Silicon crystals with other elements such as Boron, Phosphorus or Arsenic—incidentally, the latter being the same elements as those used for the EtMe3Z+ cations—leads to materials with different polarity. The combination of these polar materials in a single device, the bipolar transistor, led to the microelectronics revolution of the 20th century. Thus, the utilization of anion radical salts with slightly different cations, and hence very different electronic states in a single device, could lead to similarly exciting developments. Whether there will be new physics, new technology or both, it will be thrilling to observe the progress in the field.
References
- 1. Kato, R., Tajima, A., Nakao, A. & Tamura, M. Two pressure-induced superconducting anion radical salts exhibiting different spin states at ambient pressure. Journal of the American Chemical Society 128, 10016–10017 (2006). doi: 10.1021/ja063525l
About the Researcher
Reizo Kato
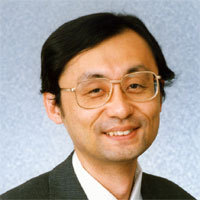
Reizo Kato was born in 1955 in Yamaguchi, Japan. He received his BSc degree in 1979, MSc in 1981 and DSc in 1984 from the University of Tokyo. He was appointed as a research associate of the Department of Chemistry at Toho University in 1984, and promoted to lecturer in 1988. He joined the Institute for Solid State Physics, the University of Tokyo, as an associate professor in 1990. Since 1999 he has been the chief scientist and director of the Condensed Molecular Materials Laboratory at RIKEN. He received the Chemical Society of Japan Award for Young Chemists in 1990 and the IBM Japan Science Prize in 1995 for his work on molecular conductors. His research has focused on the development of new molecular materials, especially molecular metals and superconductors.