Mar. 19, 2009 Research Highlight Chemistry
Seeing molecules move in real-time
Ultrafast lasers instantaneously track a molecular twist in progress
Although chemists are experts at characterizing the products of their reactions, understanding what goes on inside the beaker—where atoms twist, bend, separate, and rejoin into new molecular units—remains a mystery on many levels.
“Watching chemical reactions in real-time has long been a dream of chemists,” says Tahei Tahara of RIKEN’s Advanced Science Institute in Wako. “To reach a correct understanding of chemical reactions, this ‘watching’ is crucial.”
Now, thanks to Tahara and his colleagues, scientists are awakening to a reality where an atom can be followed along a three-dimensional path. In their latest work, Tahara and a team of international and Japanese scientists have directly observed how an organic molecule named stilbene rearranges its structure1.
Good vibrations
The best way to watch reactions in real-time is to monitor molecular vibrations. When light hits a molecule, it can interact with the chemical bonds between nuclei and cause them to vibrate periodically. These vibrational frequencies are chemically specific: for example, a carbon–carbon bond vibration is easily distinguished from a carbon–hydrogen signal. And, because very small changes to a chemical structure create easily observed shifts in vibrational frequencies, tracking these motions provides a way to follow the dynamics of a reaction.
However, tracking atoms in chemical reactions has previously seemed unrealistic, because the nuclei move so fast—movements can be complete within 1 picosecond (10-12 second). Polyatomic molecules like stilbene are even more challenging to visualize because scientists must account for multiple vibrations. Furthermore, some characteristic vibrations used for chemical identification often occur at low frequencies that are beyond the limits of most analytical instruments.
Probing atoms with ultrafast light
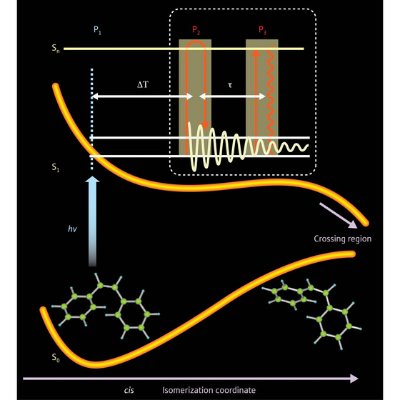
The development of lasers that emit light faster than nuclei move allowed scientists to make real-time observations of chemical reactions. Initial experiments used a ‘pump–probe’ technique: a first laser pulse—the pump—excited the molecule to initiate a reaction, and then the second pulse—the probe—captured the position of the nuclei. Changing the time delay between pump and probe pulses allowed pictures of the chemical reaction to emerge.
The resolution obtained with the pump–probe technique, however, is limited. In 2003, Tahara and his colleagues developed a new technique that combined pump–probe methods with Raman vibrational spectroscopy to measure the movements of short-lived, excited-state molecules2.
The researchers then initiated a chemical reaction by exciting electrons with a laser pulse. After a short time delay, they induced the entire molecule to vibrate using 10-femtosecond (10-14 second) lasers. Finally, using a third laser pulse, they measured how these atoms vibrated in real time, as the reaction progressed (Fig. 1).
Tahara and his team’s work allowed the low frequency motions in the excited state to be accurately resolved, which provides information not accessible by other techniques, and is crucial for understanding how a polyatomic molecule like stilbene can rearrange its structure.
Capturing a twist in progress
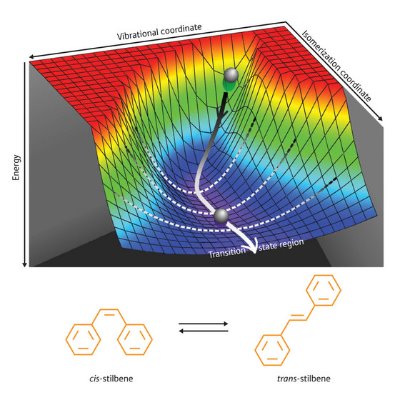
Stilbene is one of the most popular molecules used to study photoisomerization, the class of reactions that powers molecular switches and plays a central role in vision. The initial isomer, called cis-stilbene, has two benzene rings positioned close together and connected by a carbon double bond. When excited by light, this molecule twists and rearranges to trans-stilbene, such that the benzene rings end up far apart.
Scientists have long believed that the rearrangement of stilbene is accomplished by large vibrational movements of the benzene rings. Watching this reaction with Tahara’s spectroscopic method, however, revealed that the molecule changes geometry using a completely different mechanism.
“With excitation, the central carbon double bond is weakened,” explains Tahara. And then, hydrogen atoms attached to the carbon double bond move in opposite directions, initiating a twisting motion that leads to trans-stilbene. “That stilbene twisting is realized by hydrogen atom movement, [and] not by a large motion of benzene rings, was surprising to us,” says Tahara.
Computer-aided pictures
To visualize the three-dimensional molecular motion (Fig. 2), the team combined experimental results with a high-level quantum-chemical calculation. The computation correlated the frequency changes observed in the experiment with particular molecular movements—and helped identify the exact twisting mechanism that had previously eluded researchers.
“Quantum computation was indispensable in this work,” says Tahara. “However, we had to be very careful to choose the most reliable computational method because different methods provided significantly different computational results.”
Fortunately, Tahara and his team’s experiments provided reliable data that they could use to gauge the accuracy of theoretical calculations—a combined approach that will be useful in visualizing other molecular systems.
“Because it is dangerous to blindly believe the computation, it is crucial to check the consistency between the experimental data and computation, especially for calculations on excited polyatomic molecules,” explains Tahara. “We carefully examined the reliability of different methods by comparing the computational results with the spectroscopic data of stilbene.”
“In a sense, the data supplied by this type of advanced spectroscopy provides check points for the theoretical calculations that are now advancing very rapidly,” he says.
Lasers or test tubes?
With scientists now able to observe what goes on in their beakers using ultrafast lasers, it begs the question—can they start to use those lasers to control chemical reactions in ways never seen before?
Tahara says he doesn’t know how conceivable it is to control chemical reactions by light. “Nevertheless, I would like to try it on the basis of solid understanding of the potential energy of reactive molecules, which is obtainable by this type of study.”
References
- 1. Takeuchi, S., Ruhman, S., Tsuneda, T., Chiba M., Taketsugu T. & Tahara, T. Spectroscopic tracking of structural evolution in ultrafast stilbene photoisomerization. Science 322, 1073–1077 (2008). doi: 10.1126/science.1160902
- 2. Fujiyoshi, S., Takeuchi, S. & Tahara, T. Time-resolved impulsive stimulated Raman scattering from excited-state polyatomic molecules in solution. Journal of Physical Chemistry A 107, 494–500 (2003). doi: 10.1021/jp0270856
About the Researcher
Tahei Tahara
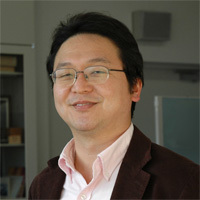
Tahei Tahara was born in 1961 in Tokyo, Japan. He graduated from the Faculty of Science, the University of Tokyo, in 1984, and obtained his PhD degree in 1989 from the same university. He became research associate of the Department of Chemistry, Faculty of Science, the University of Tokyo in 1989, and then moved to newly founded Kanagawa Academy of Science and Technology (KAST) as research associate in 1990. In 1995, he joined Institute for Molecular Science (IMS) as associate professor and started his own research group. He was appointed to Chief Scientist of RIKEN in 2001 and, since then, he has been director of Molecular Spectroscopy Laboratory. His research interest is development and application of advanced molecular spectroscopy to study dynamics and structure of 'complicated' molecular systems in the condensed phase. He currently focuses on ultrafast spectroscopy and nonlinear spectroscopy in solutions, at interfaces, and for micro-spaces.