Aug. 17, 2007 Research Highlight Physics / Astronomy
Tuning into quantum computers
Improved device design enables the tunable interaction between the elements of a quantum computer
For fast and efficient operation of quantum computers, control of the interaction between their components, the quantum bits (qubits), is necessary. Now, researchers from RIKEN’s Frontier Research System, Wako, the Japan Science and Technology Institute, NEC Corporation and the US Massachusetts Institute of Technology have developed an architecture that allows tunable control over the qubits of a superconducting quantum computer. Their study represents a large step towards the realization of large-scale quantum computers with controllable qubit interactions.
Quantum computers are different
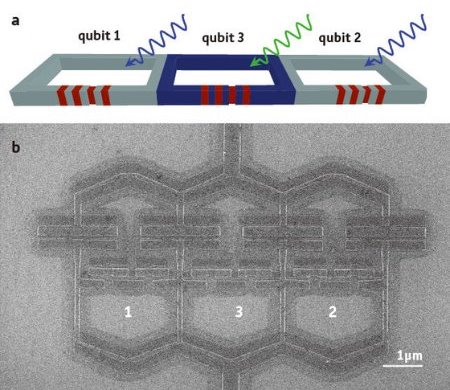
Compared with conventional computers, quantum computers hold great promise as a much faster alternative for solving certain mathematical problems, such as the simulation of quantum-mechanical systems. Another example is the factorization of integer numbers into the products of prime numbers—an operation that would require a huge effort by conventional computers and would take considerably longer to complete. The interaction between the qubits gives quantum computers their unique attributes, and it would take a significantly larger number of bits from conventional computers to even simulate the operation of a quantum computer.
There are a number of possible designs for quantum computers. One example uses atoms cooled to very low temperatures. For this, the atoms are suspended in vacuum and lined up by complex electromagnetic fields. Although larger systems of qubits composed of atoms have been successfully demonstrated, such schemes remain relatively cumbersome. On the other hand, solid-state based systems offer desirable ease of fabrication—echoing the success of conventional silicon-based computing—and are therefore intensively pursued worldwide.
A representative type of qubit for solid-state based quantum computers is formed by loops of superconducting rings made from aluminum (Fig. 1). At certain points the rings are made insulating through oxidation of the aluminum, which creates so-called Josephson junctions. Tiny magnetic fields within these rings then are able to generate electrical currents across these Josephson junctions.
When exposed to the right magnetic fields, a stable situation is reached that balances the current flowing clockwise and anti-clockwise through the rings. This renders the rings insensitive to small variations in the magnetic field and optimizes their use as qubits. Of importance to the performance of quantum-computing tasks is the quantum-mechanical coupling of neighboring rings.
Controlling coupling between qubits
While the interaction between the qubits of a quantum computer is important, good control over this coupling is essential. “Tunable coupling, in particular coupling which can be switched off completely, is highly desirable for implementing large-scale quantum computing,” comments Yasunobu Nakamura from the RIKEN team. Turning off the coupling prevents undesirable interaction between the qubits. Otherwise, as Nakamura points out, “the qubits would interact with each other all the time and even a simple decomposition of the signal would be difficult.” Cumbersome architectures would be required to compensate for such undesired side effects.
Therefore, the team’s demonstration of tunable coupling between the qubits of such a superconducting quantum computer, as reported in the journal Science 1, represents an important step towards the realization of complex quantum computers.
In this approach, tunable coupling is based on two qubits that are linked via a third and passive qubit that mediates the interaction between the active qubits (Fig. 1a). Importantly, the internal energy scale of each of the three qubits is different. While the two active qubits have only marginally different energy spectra, the mediating passive qubit has a larger energy scale. Microwave radiation at selected energies then provides deliberate and controllable coupling between the energy states of the active qubits, whereas the energetic states of the passive qubit remain unaffected due to the large energy difference. Therefore, the ‘coupler qubit’ merely mediates the coupling between the ‘active’ qubits. The microwave radiation is fed to the qubits via an on-chip transmission line, which is readily scalable to a higher number of qubits.
Detecting hackers
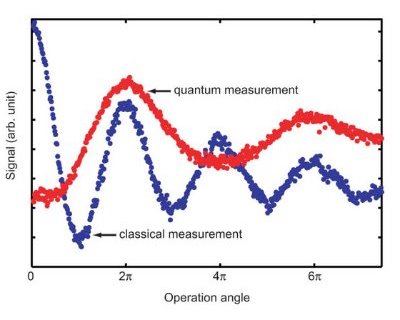
To experimentally apply the new design concept, the researchers implemented a simple quantum computing protocol to determine whether the system could detect a computer hacker attempting to modify the system. With a classical computer, such infiltrations are impossible to detect.
If the hacker altered the quantum state of the coupled qubits by using the same tunable interaction described above, it would lead to a periodic modulation of the quantum state with a periodicity of 4π inherent to such quantum-mechanical manipulation. However, classical measurements cannot detect the subtle change and results in observation of a 2π periodicity.
The test measurements performed by the researchers are shown in Figure 2. In an experiment simulating a classical computer, the 2π periodicity is seen (blue curve) and they could not distinguish whether the hacker had modified the state or not. However, the quantum measurement (red curve) clearly reflects the doubled 4π period, which clearly indicates the presence of the hacker.
Large-scale quantum computation
These experiments are an important demonstration of the principle, yet further improvements are needed. For example, longer lifetimes of the quantum states would allow for better and more complex quantum operations. These longer lifetimes can be achieved by improving the stability of the quantum states towards, for example, fluctuations in the external magnetic field.
This example of microelectronics technology by the team shows that large-scale integration requires precise control of each individual element. Indeed, Nakamura points out that the team “hopes to demonstrate that we can precisely control and characterize the quantum state of a large-scale artificial quantum system.” Eventually, such improvements could lead to the first large-scale quantum computer.
References
- 1. Niskanen, A. O., Harrabi, K., Yoshihara, F., Nakamura, Y., Lloyd, S. & Tsai, J. S. Quantum coherent tunable coupling of superconducting qubits. Science 316, 723–726 (2007). doi: 10.1126/science.1141324
About the Researcher
Yasunobu Nakamura
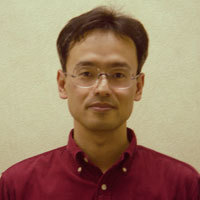
Yasunobu Nakamura was born in Osaka, Japan, in 1968. He graduated from the Faculty of Engineering, University of Tokyo, in 1990, and obtained an MSc in 1992 from the Superconductivity Research Course of the same university. Immediately after that, he joined NEC corporation, in Tsukuba, Japan, where he started his career in mesoscopic physics. Since the late 1990s he has been working on superconducting devices for quantum information processing. Nakamura also spent one year as a guest researcher at TU Delft in Delft, the Netherlands, from 2001 to 2002. Now he is a research fellow at the Nano Electronics Research Laboratories of NEC Corporation as well as a researcher at the RIKEN Frontier Research System.