Dec. 13, 2013 Perspectives Engineering
Electronics, energy and emergence
Yoshinori Tokura, Director of the RIKEN Center for Emergent Matter Science, explains recent advances in emergent matter science.
Yoshinori Tokura
Director
RIKEN Center for Emergent Matter Science
Emergent matter science is a new and rapidly growing area of research that seeks to understand and exploit the unusual phenomena that arise when materials or forces are combined in novel ways. The field draws on a diverse range of disciplines, including condensed-matter physics, materials science and quantum physics, as well as the exciting discoveries that occur at the interface of these areas.
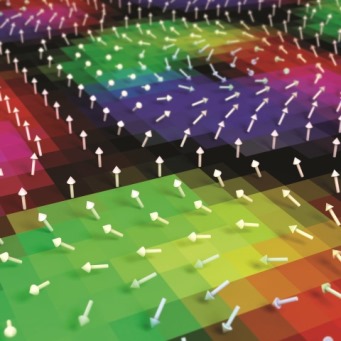
Modern computers, made possible by the semiconductor revolution of the 1950s and 1960s, are growing ever faster and more powerful. However, despite rapid changes in performance, the fundamental technology that underpins computers has changed surprisingly little since the invention of integrated circuitry over half a century ago.
More recently, the increasing demand for faster and more compact processors has pushed conventional systems to their physical limit. Additionally, the significant power requirements of advanced circuit boards have made improving their energy efficiency an enormous scientific and technical challenge for the microelectronics industry. In response, emergent matter scientists are re-examining the fundamental properties of materials and the ways in which they interact with heat, light, electricity and magnetism—paving the way for faster computers, superior methods for data storage and an improved flow of information around circuits.
Building better switches
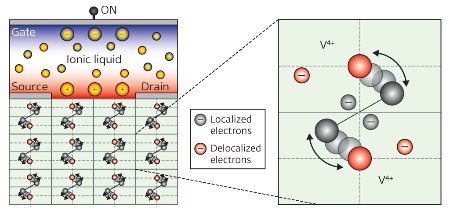
A promising area of emergent matter science involves strongly correlated electron systems. Although metals generally behave as if their electrons diffuse freely through a continuous sea of electrical charges, in some materials these conducting electrons are ‘locked’ into specific positions around atoms. This phenomenon, known as ‘strong correlation,’ affects the properties of materials in unusual ways—for example, electrical insulators can become metallic conductors by applying just enough heat or pressure to slightly change their atomic structure. Since the two states are typically very close in energy, it only takes a small nudge to switch from one to the other, something that researchers could exploit to create microelectronic components that draw very little power.
In some cases, a magnetic field induces a similar transformation. Dubbed ‘colossal magnetoresistance,’ this effect can be used to store data by switching tiny regions of a material into either a metallic or insulating form. This type of non-volatile memory, which stores data even in the absence of a power supply, is read by measuring whether a current flows through the region. The exact mechanism governing this effect at the nanometer scale and its elucidation are of great importance to researchers, including those at RIKEN, who are engaged in developing microelectronic technologies.
Another class of important materials, known as Mott insulators, change from insulators to conductors when exposed to a small voltage. Equivalent to melting an ‘electron ice’—where electrons are locked in place—into an ‘electron liquid,’ this property allows a current to flow, meaning that Mott insulators could be used as low-power transistors. Scientists at RIKEN have made significant contributions to this area through their discovery of how the change from insulator to conductor works at the atomic scale in vanadium oxide-based systems1 (Fig. 1).
Resistance is useless
In conventional materials, an electric current typically encounters internal resistance, which leads to the generation of heat with a resulting loss of energy. Superconducting materials, conversely, conduct electricity without resistance. Yet superconductivity only arises in certain specialized materials, and then only at very low temperatures, making these systems impractical for most computers.
Materials in which electrons can flow down ‘electron highways,’ and consequently experience very little resistance, offer an attractive alternative. The leading candidates are the so-called topological insulators—materials that mostly insulate but conduct very well at their surfaces. While the generation of topological currents usually requires a strong magnetic field, in theory topological insulators can conduct electricity with little resistance at room temperature and without the need for a large magnetic field. Conductivity at the surface could also be switched on or off with a very small stimulus.
A common method for establishing a topological current relies on the Hall effect, a phenomenon in which a magnetic field applied at right angles to a stream of moving electrons creates a voltage difference between opposite surfaces of the material. Systems developed at RIKEN, in which a spontaneous Hall effect helps current to flow along the edges of the material at low temperatures, have played a significant role in advancing the field. The protocol has been successfully demonstrated under low-temperature conditions, and the next step will be to develop systems in which this behavior is sustained at room temperature.
Magnetic tornadoes
Conventionally, data is carried using electric charges. However, an alternative method involves the use of swirling magnetic vortices, known as skyrmions, which result from the self-organization of magnetic moments in ferromagnetic materials. Skyrmions form when concentric rings of atomic magnets in the atoms of ferromagnetic systems become progressively more tilted, until the magnetic moments at the very center point in the opposite direction to those in the wider bulk of the material. These alignments—just tens of nanometers wide—behave like individual particles and can move through a material as a tornado twists its way across the land.
An understanding of how skyrmions behave, and how they might be manipulated, lies at the heart of progress in the field. RIKEN has made significant progress in predicting the mechanisms that govern skyrmion dynamics, such as by showing how their motion can be driven by tiny electrical currents2. This discovery may offer a low-energy way to transport information encoded in skyrmions. The next challenge will be to overcome a fundamental problem: as skyrmions move faster under higher current densities, a low-energy skyrmion-based computer would be accompanied by slower processing speeds.
Quantum bits
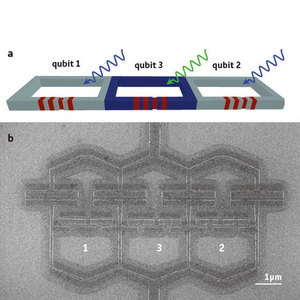
While many methods aimed at boosting the processing power of computers focus on the development of new and more efficient materials, another approach, termed ‘quantum computing,’ aims to tackle the fundamental way in which data is manipulated. In standard computers, data exists as a series of ‘ones’ and ‘zeros’ encoded by binary switches that are either on or off. However, in quantum computers data is stored in quantum bits or ‘qubits’ that exist as a combination of both states—known as a superposition. Multiple qubits can be linked by another quantum mechanical process called entanglement, and thus changing one qubit affects the whole group. Together, superposition and entanglement allow an array of qubits to access vastly more states than the same number of conventional bits, which increases the overall computing power of the system.
Researchers at RIKEN are at the forefront of efforts to create and control such qubits. One of the main challenges remains how to maintain the quantum coherence of qubits to avoid the loss of stored information due to interactions with their environment. Potential systems for exploiting qubits include the electron spins in semiconductor quantum dots and the charges and magnetic fluxes of superconducting circuits. Research is also focusing on correcting the errors that arise from quantum decoherence—a key issue for facilitating the scale-up of qubit systems to enable reliable and efficient quantum computing.
Research into quantum bits, skyrmions and topological currents has already provided a number of important advances in the quest for energy-saving applications. Before new forms of electronic devices can be developed, gaps that remain in our understanding of the fundamental issues in materials science, such as the behavior of electrons in solid matter, must be filled. These endeavors, and the research that will be carried out over the coming decade, will doubtless spark new concepts for electronic materials.
References
- 1. Nakano, M., Shibuya, K., Okuyama, D., Hatano, T., Ono, S., Kawasaki, M., Iwasa, Y. & Tokura, Y. Collective bulk carrier delocalization driven by electrostatic surface charge carrier accumulation. Nature 487, 459–462 (2012). doi: 10.1038/nature11296
- 2. Seki, S., Yu, X. Z., Ishiwata, S. & Tokura, Y. Observation of skyrmions in a multiferroic material. Science 336, 198–201 (2012) doi: 10.1126/science.1214143